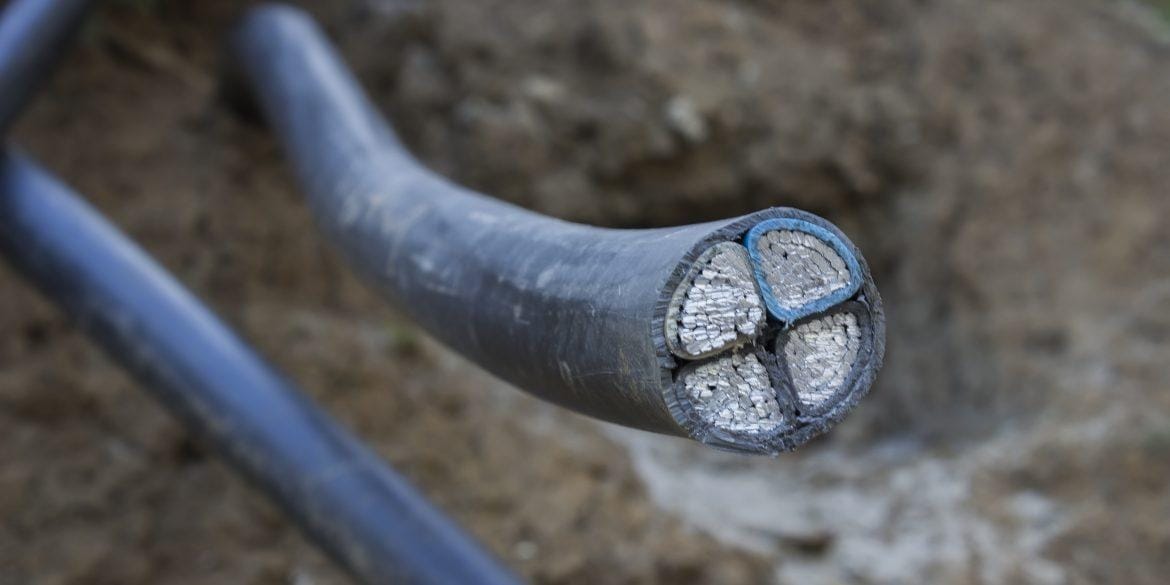
Table of Contents
Factors Affecting Cable Life Expectancy
Most power cables have a design life of between 20 to 30 years. If the cables are not fully loaded, they are expected to last beyond their design life. Power cables are available with special high-grade XLPE or EPR insulation with design lifetimes of up to 50 years. How do you calculate the expected design life of power cables for specific conditions? Well, many factors determine the life of a cable, and the theories have progressed significantly since the simplistic Montsinger rule was proposed, so it can be challenging.
Many factors can cause aging and hence affect the lifetime of extruded insulated power cables, and these fall under the categories of physical, chemical, and electrical. In general, the insulation is the weakest part of a cable, and the electrothermal stress due to voltage and temperature is the main stress on cable insulation in service. These stresses cause aging, which refers to non-reversible changes in the properties of the insulating materials and components of a cable system due to the in-service operation or testing of cables.
The stresses on a cable system that impact the expected life of the cable insulation are:
- Thermal stress – due to the heating from the current flowing in the conductors.
- Electrical stress – due to the applied electric field.
- Mechanical stress – static (bending and compressive) or dynamic (vibrations) forces.
- Environmental – such as ambient temperature, humidity, corrosive environments, pollution, and exposure to solar radiation.
The stresses of most concern, since these are the stresses which mostly age and cause the failure of the electrical insulation, are the thermal and electrical stresses. Note that low temperatures can be as harmful as high temperatures because the insulation tends to freeze and crack.
What Happens as Cables Age?
Several chemical changes occur in electrical insulating materials with time under conditions of elevated temperatures and applied voltages [1]. The primary form of deterioration of cable insulation introduces acid groups into the polymeric or liquid insulation, which is slow oxidization that increases the conductivity and power factor of the insulation. The oxidization also splits the polymer chains in resin insulation and decreases its tensile strength.
Another form of deterioration for plastic insulation is the stiffening and brittle hardening due to plasticizers and excessive oxygen cross-linking of polymer chains. Over time the lighter-weight substances inside the insulation, which render it flexible, will slowly evaporate. The introduction of oxygen from the environment also increases the cohesion between neighboring molecules, while simultaneously breaking them and making them shorter, with the net result being an increase in hardness and reductions in the strength to bending and stretching.
The final form of deterioration inside cable insulation is the purely thermal or internally catalyzed depolymerization of plastic insulation (in fact, this breakdown mechanism affects all polymers). This form is due to elevated temperature that causes polymer chains to break down into shorter sections.
Montsinger’s Rule
During the period of the 1930s, whilst insulation lifespans were beginning to be studied, the ‘Montstinger rule’, was proposed as follows:
Insulation life is halved by a temperature increase of 8 to 10 ˚C.
This ‘rule’ is still, in various forms, in published use to this day but it is important to understand it was originally proposed by chemists to provide rough approximations of the temperature coefficients of the reaction rates during chemical breakdown caused by thermal stress. It is also theoretically not possible to apply this rule of 8 to 10 degrees below 115 ˚C, where the rule changes to about a 5-degree rule [2].
Thermal Stress
The heat generated inside power cables increases their operating temperature above that of ambient temperature, which gives rise to thermal stress. Increased temperatures result in increased chemical reactions within the insulation and cause aging. The aging caused by thermal stress is highly dependent on the relative current loading of the cable and the insulating material. For XLPE insulation, the thermal aging at elevated temperatures causes a considerable reduction in breakdown strength, however, that of EPR insulation is significantly less affected.
The maximum permissible operating temperatures provided by the standards are within the temperature threshold for both steady-state and overload or short-circuit operation. An overload temperature of 105 ˚C for XLPE insulation is a safe limit, and that of EPR insulation may be slightly higher again.
The Arrhenius Equation
The Arrhenius method is the most widely used for predicting the aging of electrical insulating materials of power system equipment. The Arrhenius approach is best applied to characterize one failure mechanism at a time, most commonly thermo-oxidative degradation of cable insulation.
The Arrhenius equation describes a specific rate constant (K) as a function of activation energy (Ea) for the reaction, the Boltzmann constant (KB), and temperature (T).
K = Ae-[Ea/KBT] (Equation 1)
The activation energy value is obtained through measurement by physical experiments. Temperatures within 25 °C of the maximum service temperature for a material are recommended, but this is not easy when obtaining the activation energy of mechanisms over long lifespans such as 40 to 50 years.
A useful form of the Arrhenius equation for estimating the lifetime of cables based on thermal stress is:
L = taging*e^(Ea/KB*(1/Tcond-1/Taging)) (Equation 2)
Where:
L is the predicted cable lifetime (hours).
taging is the time (hours) for the cable insulation to fail (for the chosen failure mechanism).
Tcond is the cable operating temperature (Kelvin).
Taging is the temperature (Kelvin) at which a failure occurs (for the chosen failure mechanism).
Example Calculation using the Arrhenius Equation
The Arrhenius equation will be used to perform an estimated cable lifetime calculation.
The cable system-related input data is as follows: Cable primary insulation material = XLPE with normal operating temperature rated at 90 ˚C.
Activation energy (Ea) = 1.13 electron Volt (eV) at failure being 60 % retained relative elongation and calculated from presented Arrhenius-type plots.
Oven aging test results = 504 hours to 150 ˚C.
Therefore, the estimated lifetime is as follows:
L = 504 * e ^ ((1.13/8.61733326E-5)*((1/(90+273.15))-(1/(150+273.15))) = 84343.44 hours = 9.622 years.
As can be seen from the example calculation there are two main problems with the Arrhenius method these are:
(1) That it is overly simplistic and only considers thermal stress and not electrical stress.
(2) While it is the preferred method to use rather than rely upon an 8 or 10-degree rule, the Arrhenius equation consistently underestimates cable life expectancy compared with observed real cable life [2].
The Arrhenius method, despite its limitations, can produce reasonably accurate estimates of power cable life expectancy but only if actual cable operating temperature (based on the load current) and ambient temperature are used and not the extreme values as seen from the example above.
Electrical Stress
There is a threshold electrical field stress above which the life curve of a cable is adversely affected. This electrical stress is the threshold voltage at which discharge may occur. It has been shown that the Weibull distribution applies to the lifetime of XLPE insulation subjected to repeated impulse voltages.
For HV cables, it is essential to achieve an undistorted radial electric field inside the insulation, so an extruded semi-conductive material is also applied to the insulation [1]. There are models available for estimating the lifetime of cables considering only the electrical stress. The better models, again, are referred to as the electrothermal models that consider both the thermal and the electrical stresses.
Load Cycling and Thermal Transients
The consideration of thermal and electrical stresses on cables affecting their life span is made difficult by the thermal transients caused by load current cycles. These transients add time dependency to the stresses on the cables while in service.
HVDC Cables
The comparison between the procedure for estimating the life of DC cables under load cycles and that for AC cables shows that the former is far more complex than the latter. For HVDC cables, the lifespan calculations must consider the electric-field inversion phenomenon of the insulation [3]. Local in-homogeneities of the extruded insulation for HVDC cables may cause localised space charges.
The major differences between HVDC cables compared with HVAC are as follows:
- Circuits are either unipolar or bipolar instead of three phases.
- No skin or proximity effects occur in the conductor.
- No dielectric losses occur in the insulation.
- No cyclic or eddy current losses occur in the outer metallic layers.
Whereas in HVAC cables, the maximum electric field and temperature always occur at the conductor-insulation interface, in HVDC cables, the outer section of the insulation is the most electrically-stressed part. This difference in HVDC insulation is because the electric field in DC cables depends on the voltage, the electric and thermal resistivity of the dielectric, and the insulation temperature drop, which, in turn, is proportional to the square of load current. When an HVDC cable is loaded, the electric-field profile in the insulation changes significantly, which leads to the DC field inversion phenomenon.
Power Quality Impact
The use of power electronic equipment such as AC/DC converters injects harmonic currents into electrical power networks, which may distort the voltages. The voltage distortion that arises can increase the peak or the RMS value as well as the rate of rise of the voltage compared with sinusoidal conditions. It is the increase in the voltage peak that is mostly responsible for accelerating the aging of electrical insulation [4].
External Environment
Higher current loads and higher ambient temperatures both have an accelerating effect on the aging of cables. In general, the exposure of cables to water or other contamination sources, such as oils and sulfur, has not been shown to reduce cable lifespan [5].
For cables, without a metallic sheath, the ingress of water is delayed but inevitable. The water will eventually reach the insulation through diffusion, which is material and temperature-dependent. The rate of permeability through a polymer increases when the temperature increases. Moisture inside a power cable can degrade the insulation, trigger water trees, and reduce life expectancy. On the other hand, cables that include a solid metallic (water-blocking) sheath may be considered a dry construction.
For submarine cables, an accelerated age test is required to prove that the insulation, when saturated with seawater, will maintain adequate dielectric strength. Most widely accepted wet age testing protocols are at either 50 Hz or 60 Hz and up to 2 years duration. The reason the water tests are required is to prove that the relevant water barriers are functioning correctly [6].
Corrosion of cable installed in wet environments especially is also of high concern. The corrosion caused by seawater is more aggressive than that in soils. For a dry cable construction, corrosion should be of little to no concern so long as the cables have been tested to be resistant to water ingress and the cables have been installed correctly. Whereas the corrosion rate of ferrous metals (for example, mild steel and stainless steel) decreases with depth, the corrosion rate of non-ferrous metals, such as aluminium (commonly used in cable constructions), increases with depth despite the lower temperatures.
Cables with Recycled Insulation
The degradation of cables constructed using new PVC compared with recycled PVC is generally caused by the same dehydrochlorination hydrogen reaction. Recycled PVC has a shorter thermal lifespan, at approximately 6.3 years at the upper-temperature limit of 60 degrees s, compared with 30 years for new PVC. Furthermore, cable insulation with higher concentrations of polyethylene may have an even shorter lifespan than 6.3 years [7].
References
[1] Dakin, T., “Electrical Insulation Deterioration Treated as a Chemical Rate Phenomen”, AIEEE Transactions 1948.
[2] Clark, F.,M., “Factors affecting the mechanical deterioration of cellulose insulation”, AIEE Transactions 1942.
[3] Mazzanti, G., “Life Estimation of HVDC Cables Under the Time-Varying Electrothermal Stress Associated with Load Cycles”, IEEE Transactions on Power Delivery, 2015.
[4] Pacheco, C.,R., et. al., “Power Quality Impact on Thermal Behaviour and Life Expectancy of Insulated Cables”, IEEE Transactions, 2000.
[5] Papadopulos, S., “Consideration of ageing factors in extruded insulation cables and accessories”, GT 21.09, CIGRE 1992.
[6] CIGRE TB 722, “Recommendation for additional testing for submarine cables from 6kV up to 60kV”, Working Group B1.55, April 2018.
[7] Yukawa, H., et. al., “A10.2 Development of recyclable low-voltage insulated cable”, Jicable Conference 1999.